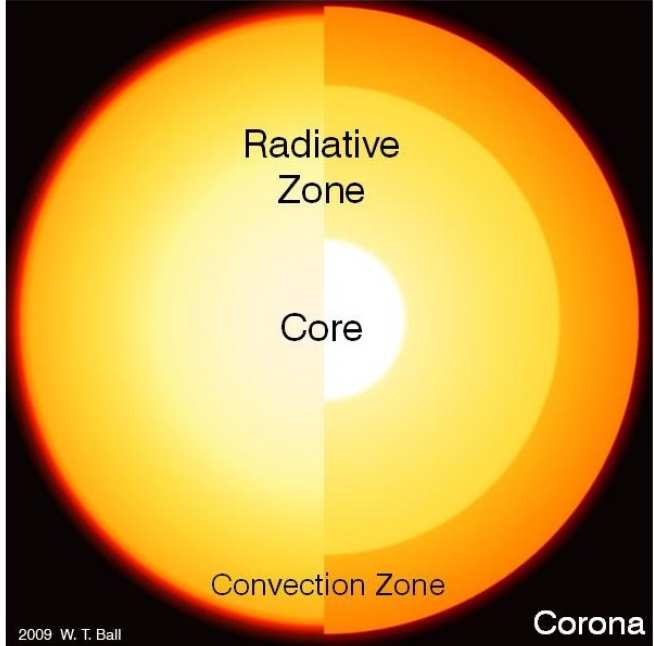
While the Sun is simple in essence, being a giant ball of hydrogen, helium and a few trace elements, it displays highly dynamic and chaotic behaviour.
Internally, the Sun is composed entirely of plasma and is divided into two distinct regions. The radiative zone (see Figure 1), containing the fusion core, extends out to ~70% of the solar radius and exhibits solid body rotation, while photons escape the core by random walk. Beyond this region, convection becomes the driving force for energy distribution and is active from the top of the radiative zone to the photosphere (the effective surface), where it manifests itself as granulation. The convection zone undergoes differential rotation by both latitude and depth, rotating more slowly at the poles than at the equator (~27 days). It is the combination of charged particles in the plasma and differential rotation that is key to building the magnetic field.
Fig. 1: A schematic diagram of the interior of the Sun. All energy is produced in the core, is radiated through the radiative zone, convects to the surface in the convection zone and is released into space at the photosphere. The corona extends away from the surface as a tenous atmosphere.
Turbulent flow in the convection zone combined with electric fields within the plasma produce magnetic fields which in turn further generate electric fields. Helioseismology has determined that there is a significant shear profile at the boundary layer between the convective and radiative zones, known as the tachocline. Initially, the magnetic field is polodial (poleward) in nature.
Differential rotation winds the magnetic field lines around the equator, stretching and strengthening them in the process - this is known as the omega effect (Figure 2a). Convective material overshooting to the base of the tachocline is stretched by the rapidly changing rotation rate and strengthens the polodial field into a toroidal field (along latitude lines). These boosted magnetic fields, embedded within convection currents, rise up through the convection zone and are twisted as a result of the turbulence and varying rotation rate - the alpha effect (Figure 2b). Eventually these field lines breach the surface, manifesting as many different features, most obviously sunspots.
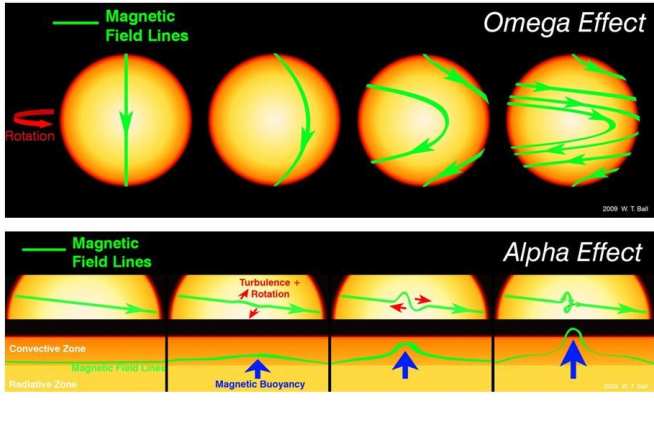
Fig. 2: Two processes involved in the winding up and boosting of magnetic fields in the Sun: the omega (2a, top) and alpha effects (2b, bottom). All field lines are sub-surface except for where shown in bottom half of 2b.
Sunspots consist of a darker region at the centre, the umbra, surrounded by the penumbra. Temperatures within umbrae range from 3900 - 4800 K and within penumbrae from 5300 - 5500 K, much less than the effective temperature of the quiet sun (regions free of magnetic features) at 5777 K. Pores are simply sunspots without the penumbral region and will turn into sunspots when a strongly inclined magnetic field at their edge becomes unstable and interacts with the photospheric medium, forming a penumbra. Beyond this, with an increasing magnetic field, sunspots can expand to sizes in excess of 40 Mm across. These features are shown in Figure 3 below.

Fig. 3: An active region on the solar surface, from Rouppe van der Voort et al., 2005, taken with the Swedish Solar Telescope. A sunspot in the top left is compose of two distinct regions: the dark umbra and the lighter surrounding penumbra. An penumbra-free pore is in the bottom right. Granulation is clearly visible as the mottled effect across the surface.
As the underlying cause of all the properties observed within sunspots the link between with magnetism cannot be understated. The magnetic field strength within sunspot umbral regions can range from 1500 - 3500 G and in pores from 1600 - 2100 G. In comparison, the quiet sun exhibits a uniform magnetic field of approximately 20 G, though it is likely that this may be confined to smaller, unresolved areas. Comparing the magnetic field strength and the continuum intensity for regions within dark magnetic features indicates an inverse relationship. The basic idea that follows is that magnetic fields inhibit convection motion of granules (and therefore energy transport) as a function of its strength. Thus, regions of higher field strength are darker. This is not the case for all photospheric magnetic features, such as faculae.
Faculae are magnetic features brighter than the quiet sun. When the Sun is most active their influence on energy output outweights the effect of sunspots. While faculae appear as large, continuous regions on full disk images, high resolution images reveal they are composed of small brightenings (see Figure 4).
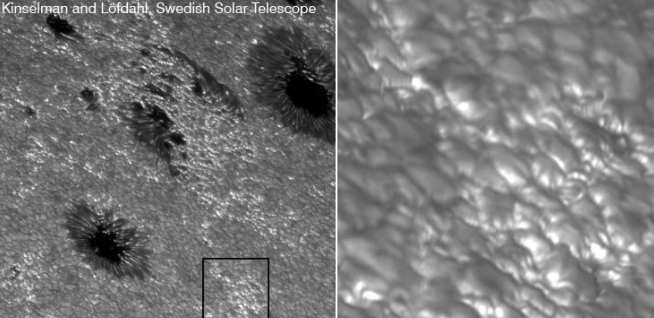
Fig. 4: An active region, taken by Kinselman and Lofdahl using the Swedish Solar Telescope. The bright regions on the left appear as discrete small-scale brightenings at high resolution (right).
Spruit, in 1976, was the first to describe these features: small, vertical columns located on the edge of granules, known as flux tubes, evacuated and thus made optically thin from a strong magnetic field on the order of 1500 G. This can be seen in Figure 5 where the thin layer bewteen the granule wall and magnetic boundary (dotted line) is optically thinner on the edge of the granule than on top and so is hotter and brighter. Radiation flows in from the hot walls and heats the inner tube to above that of the surrounding quiet sun.
Fig. 5: Schematic of magnetic flux tube, from Keller et al., 2004. Facular brightening originates in the granule wall and where the optical thickness is low (grey line). Looking into the downward convective region at an angle increases the visible area of the hot wall making faculae more prominent away from disk centre.

There is a network of > 1 kG field flux tubes located at granular boundaries and an intra-network magnetic flux within of very low magnetic field strength close to zero. Network elements consists of tiny, unresolvable flux tubes, like those of faculae. Unlike faculae, though, they are always brigther than the background quiet sun and their contrast remains relatively consistent from disk centre to limb. The importance of the network in long term irradiance variations is not understood. It would be expected that the influence of network flux tubes, along with facular regions, would increase with a larger, global solar magnetic field and because the quiet sun, and therefore the network, covers the solar disk at all times, it may be an important factor in long term solar irradiance variations on both cycle and secular (long-term) time-scales. Find out more about the contribution of small scale magnetic elements.
Text by Will T. Ball, extract from thesis in work.